By: Jonah Bloch-Johnson
Hi. I’m Jonah, a scientist here at the UoR, and I study whether global warming will be more like drinking water, soda, or beer.
What do I mean by that? Let me explain.
Both thirst and the Earth’s climate are feedback systems – they react to an external nudge by either undoing or amplifying that initial push. A reaction that undoes the push is called a negative feedback, and an amplifying one a positive feedback.
For example, we all have experience being thirsty – in fact, you might be thirsty right now. How might you react? Perhaps you reach for a cold glass of water (Fig. 1). Drinking the water quenches your thirst. The initial nudge – your thirstiness – prompted you to react by doing something that got rid of your thirstiness; or put another way, the quenching nature of water provided a negative feedback.
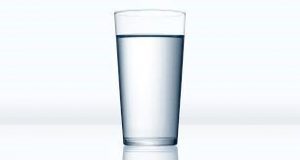
Figure 1: A glass of water: an absolute classic beverage.
But what if, instead, you reached for something sweet, like soda, or juice? Sweet drinks taste good, so that while they quench your thirst, they also stimulate you, making you want to drink more. The sweetness adds a positive feedback. The combined result is that drinking a sweet drink lessens your thirst, but not as much as just drinking water; the overall negative feedback is weaker. So you might find yourself drinking more soda than you would water. Fig. 2 shows a drink called a “Big Gulp” which is popular back in my home of the USA and contains about a litre of soda. Big Gulps of water are few and far between.

Figure 2: A Big Gulp: finally, enough soda.
Ah, but what if instead of a sweet drink, you decided to go for something with a bit of a kick? Some beer, cider, or whisky, for example (Fig. 3)? Depending on the drink, it may be more or less tasty – at first! But after a few drinks, the idea of getting another may become more appealing. In other words, with alcohol, the “tastiness” of the substance can depend on how much of it you’ve already consumed. What might start as a simple thirst can run away into a bender, as your overall feedback goes from negative to positive.

Figure 3: Beer: another round, please!
What does any of this have to do with global warming? First, a bit of science. You might have heard that energy is conserved – it can be moved around, or change forms, but is never created or destroyed. One of the ways energy moves around is as light, which carries energy from the place it is radiated to the place it is absorbed. You may be used to light as something you can see, but there are actually many colours of light beyond the range our eyes can capture. Everything is constantly giving off light, invisible and/or visible, including you and me (doesn’t that give you a bit of glow?). And I’m not talking about reflecting visible light – you give off your own light, even in the dark, which is how some snakes are able to find you in the night (!).
The hotter you are, the more light you give off – and so the more energy you radiate away. The Sun is so hot (and huge) that even though it is quite far away, a substantial amount of the light it gives off reaches us here on Earth, where we gain the energy it carries. As a result, the Earth warms up – and gives off light of its own. The additional carbon dioxide we have been adding to the air for the past century or two intercepts some of the Earth’s light that was destined for space. As a result, the Earth doesn’t lose some of the energy it would otherwise have lost. This is kind of like us getting thirsty – it is a nudge, a push to a system that was previously in balance.
How does the Earth react? That excess energy warms the Earth’s surface, and as a result, it gives off more light, offsetting the carbon dioxide’s effect. In other words, we have a negative feedback, which we call the “Planck” feedback. This warming continues until the amount of light leaving the Earth once more balances the amount entering, and the planet is no longer thirsty. If the Planck feedback was the only one in the Earth’s climate, global warming would be less of an issue, because we’d only need to warm a little to restore balance (blue line in Fig. 4).
But like with soda, there’s a sweetness to warming that keeps the Earth going further: warming also causes more water vapor to be evaporated into the atmosphere. Water vapor also intercepts some of the Earth’s light destined for space, so it causes still more energy to stick around as excess. In other words, it is a positive feedback. It is not so strong as the Planck feedback, so that warming still manages to offset the initial carbon dioxide nudge; but instead of a little warming, we get more of a Big Gulp (red line in Fig. 4). And in addition to the water vapor feedback, there are others, positive and negative, associated with changes to ice, clouds, and the vertical structure of the atmosphere.
But here’s the twist: the water vapor feedback gets stronger the more you warm. In other words, it’s more like beer than soda. And the result is that too much of a push can send your planet on a bender. That’s what happened to Venus in the distant past, when the Sun’s increasing warmth caused its climate to enter a “runaway greenhouse,” evaporating its oceans and giving it a surface temperature of around 500ºC (gray line in Fig. 4).

Figure 4: Will global warming be more like water, soda, beer, … or vodka? Here we have four scenarios for future warming, assuming we just keep digging up fossil fuels, burning them, and leaving the released carbon dioxide in the atmosphere. In the first, the only feedback operating in the climate is the strongly negative “Planck” feedback (“hotter things give off more light”), which like a quenching glass of water simply satisfies your pang of thirst; just a little warming closes the imbalance between inflows and outflows of energy (blue line). In the second, the positive water vapor feedback (“hotter planets evaporate more water, which is itself a greenhouse gas”) and others makes warming more like soda, and you might end up drinking quite a Big Gulp to satisfy your thirst (red line). In the third, the deliciousness of the water vapor feedback gets amplified the more you warm, giving your drink a kick like a nice glass (or two, or three…) of beer (tan line). This state of affairs seems to describe the response of the CanESM5 model, which warms more than if its feedbacks stayed constant (green line). Finally, a climate with a higher feedback temperature dependence – a higher alcohol content? – can end up on quite a bender (gray line). Looking at past climates suggests that we are not at risk for this particular scenario. Figure credit: Jonah Bloch-Johnson, Maria Rugenstein, Martin Stolpe, Tim Rohrschneider, Yiyu Zheng, and Jonathan Gregory.
Is the addictive nature of the water vapor feedback important for global warming? Evidence from records of the past and computer models of the future suggest the Earth is likely not close to a runaway greenhouse. However, even if we are not at risk of “drinking” away our oceans, the road to warming may be paved with tipsiness. The green line in Fig. 4 shows the warming of the Canadian Earth System Model, Version 5 (CanESM5) if we continue to increase the carbon dioxide concentration until it reaches eight times the preindustrial level in 2250, which assumes that we continue to grow using mostly fossil fuels. Using the feedbacks and feedback temperature dependence of CanESM5, we can extrapolate warming out even further (tan line). The difference between the red and tan lines – between the soda and the beer – gives you additional warming that continues to increase with time.
While scientists have long appreciated the importance of understanding how “sweet” warming is for forecasting climate change in the coming century, it is becoming clear that after 2100, climate change will also depend on the booziness of warming. While 2100 once seemed like the unimaginable future, it will soon be within the lifetime of most of the newest humans. In the meantime, we keep spewing out CO2. In response, we may find that the Earth doesn’t just warm – it might also develop a taste for it.
Reference:
Bloch-Johnson J, Pierrehumbert RT, Abbot DS (2015) Feedback temperature dependence determines the risk of high warming. Geophysical Research Letters 42:4973-4980. https://doi.org/10.1002/2015EA000154